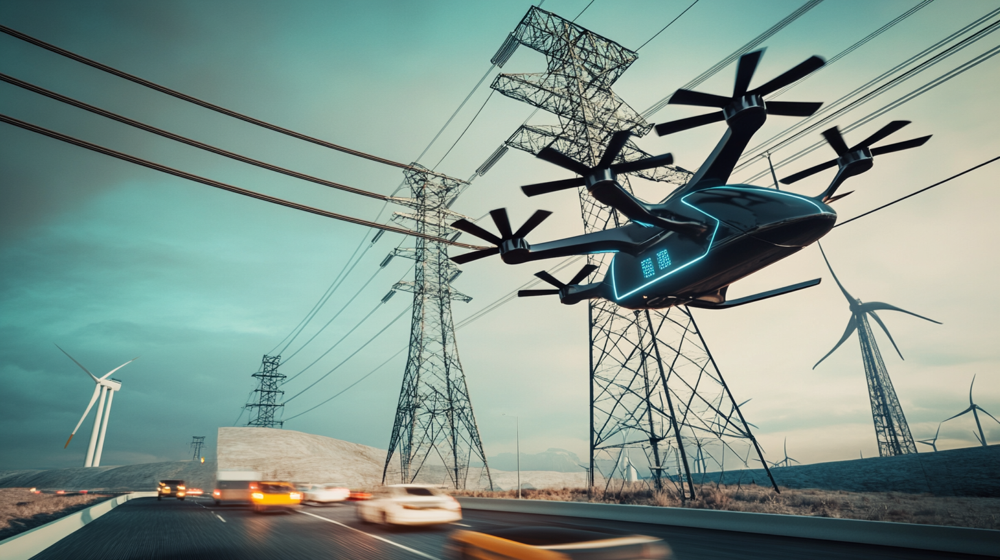
Vertiport Power Demands and Grid Infrastructure Realities
The future of advanced air mobility (AAM) relies on groundbreaking eVTOL aircraft and robust and innovative power infrastructure. Vertiports, the hubs for these electric aircraft, face significant energy challenges as they transition from concept to commercial operation.
These facilities are often likened to urban heliports with vastly greater electrical demands and require megawatt-scale power capacities comparable to small towns. Meeting these demands while balancing operational reliability, cost efficiency, and minimal community disruption is critical to the industry’s success.
Emerging technologies such as NASA’s SABERS (Solid-state Architecture Batteries for Enhanced Rechargeability and Safety) battery and microgrid solutions developed by the National Renewable Energy Laboratory (NREL) are reshaping how vertiport infrastructure is planned. However, their integration requires unprecedented collaboration among energy providers, regulators, and aviation stakeholders.
Understanding Vertiport Energy Intensity
A single vertiport’s energy consumption is a complex equation shaped by multiple variables. Baseline estimates suggest facilities will require between 1.5 and 5 megawatts (MW) of continuous power, figures that escalate dramatically during peak operational windows. For context, 5 MW can power approximately 3,500 average U.S. homes, a massive requirement condensed into aviation facilities often occupying less than two acres.
Three primary factors drive this energy intensity. First, the eVTOL charging infrastructure demands power delivery systems that rival those of heavy industrial users. Most aircraft require 300 kW to 1 MW direct current (DC) fast chargers to achieve 15–30-minute turnaround times, with prototype systems like Joby Aviation’s 1.2 MW charger pushing existing electrical hardware to its limits.
Second, operational density compounds these needs: a vertiport supporting 12 simultaneous aircraft movements could temporarily draw over 13 MW—comparable to a medium-sized data center’s peak load. Third, environmental factors introduce wild cards; cold-weather operations in cities like Chicago or Minneapolis may increase energy consumption by 15–30% due to battery heating requirements and anti-icing systems.
Real-world modeling underscores these projections. A 2024 case study of Dallas-Fort Worth vertiport proposals revealed that Benbrook Heliport’s planned expansion would require 14,319 megajoules (3.98 megawatt-hours) over a four-hour peak period—equivalent to continuously powering 400 single-family homes during those operational windows. Such concentrated demand profiles challenge decades-old assumptions about urban power distribution.
Grid Infrastructure: Costs and Upgrade Complexities
Vertiports’ concentrated energy demands pose significant challenges for existing grid infrastructure. NREL estimates that most urban distribution systems would be overloaded by 300–400% during peak operations without upgrades. Key cost components include:
Infrastructure Component | Cost Range |
---|---|
Distribution line upgrades | $8M–$16M per site |
Microgrid integration (solar + storage) | $2.1M–$4M/MW |
Transformer upgrades | $500K–$2M per site |
In addition to these costs, rural vertiports face unique hurdles, such as lacking three-phase power within two miles of potential sites, necessitating $1.2–2.8 million in distribution extensions.
Demand Charges and Peak Pricing
Unlike traditional airports with relatively stable power profiles, vertiports’ intermittent ultra-high-demand periods create financial and technical headaches. Simultaneous charging of multiple aircraft could trigger demand charges—utility fees based on peak usage—that more than double energy costs. For example, in Houston’s CenterPoint Energy service area, commercial customers pay up to $18.50 per kW for monthly peak demand above historical baselines. A vertiport drawing 10 MW for 30 minutes could incur over $185,000 in demand charges for that single spike, fundamentally altering operational economics.
Voltage Instability Risks
Concentrated 5+ MW loads pose technical risks for aging urban grids. Analysis by Pacific Northwest National Laboratory shows that sudden vertiport demand spikes could cause voltage dips exceeding 5% on distribution lines—enough to trigger protective relay operations and cascade outages. In dense metro regions like New York City, where some distribution circuits already operate at 90% capacity, vertiport interconnections may require complete circuit rebuilds to prevent neighborhood brownouts.
Prohibitive Upgrade Costs
Bringing existing infrastructure up to vertiport standards can prove financially daunting. Urban sites frequently require new substations, upgraded feeders, and reinforced transmission lines—a package averaging $3–5 million per location, according to Southern California Edison estimates. Rural vertiports face different challenges: a 2023 DOE study found 65% of potential vertiport sites in renewable energy zones lack three-phase power within two miles, necessitating $1.2–2.8 million in distribution extensions.
The National Renewable Energy Laboratory (NREL) quantifies these challenges starkly: at typical vertiport sites, existing distribution infrastructure would be overloaded by 300–400% during peak operations. This leaves developers with two equally complex paths—substantial grid reinforcement or innovative on-site mitigation.
Bridging the Power Gap: Emerging Solutions
Forward-thinking operators and utilities focus on four key strategies to align vertiport requirements with grid realities, demonstrating measurable success in the initial implementations.
Strategy | Implementation | Benefit |
---|---|---|
Microgrids | Solar PV + battery storage | Reduces peak demand charges by 40% |
Load Management | AI-powered charging scheduling | Lowers required grid capacity by 25% |
Strategic Siting | Co-location with data centers/EV hubs | Shares infrastructure costs |
Microgrid Integration
Hybrid systems combining solar photovoltaics (PV), battery storage, and backup generators are emerging as vertiport staples. BETA Technologies’ Vermont test facility pairs 800 kW solar canopies with 2.4 MWh lithium-ion batteries, reducing grid dependence by 65% during daylight operations. Such systems slash demand charges by smoothing consumption spikes, Xcel Energy’s Denver microgrid pilot achieved a 40% peak reduction through strategic battery dispatch.
Artificial Intelligence-Driven Load Management
Siemens and Skyports’ London vertiport prototype employs machine learning algorithms to optimize charging schedules based on aircraft state-of-charge, weather patterns, and utility rate structures. By staggering 1 MW charger activations within 15-minute intervals, the system lowered the required grid capacity by 25% without impacting flight operations. These AI controllers increasingly integrate with wholesale energy markets, capitalizing on real-time pricing, a capability that generated $12,000 monthly savings in a Chicago test case.
Strategic Co-Location
Locating vertiports near complementary high-demand users creates shared infrastructure opportunities. Dallas’s Vertisite initiative colocates a vertiport with an Amazon Web Services data center and public EV charging hub, splitting $8.3 million in substation upgrade costs three ways. The approach leverages overlapping demand profiles: data centers 24/7 baseload complements vertiports’ intermittent peaks, improving overall asset utilization.
Battery Innovations
NASA’s SABERS solid-state sulfur-selenium battery exemplifies the dual focus on energy density and safety required for eVTOL operations. With an energy density of 500 Wh/kg—double conventional lithium-ion batteries—and rapid discharge rates increased 50-fold through Georgia Tech collaboration, this technology enables high-frequency flight operations while mitigating fire risks. The battery’s graphene-based cathode and bipolar stacking design allow it to withstand impacts and extreme temperatures (up to 150°C), reducing the need for heavy thermal management systems.
These advancements align with vertiport energy profiles detailed in NREL’s 2024 study:
- 100 eVTOLs operating from a single site require 40–60 MWh daily—equivalent to powering 30,000 U.S. homes
- Peak demand reaches 1.5–2 MW during high-frequency operations (30+ flights/hour), comparable to 700–2,000 households
Such loads necessitate FAA-compliant infrastructure, including 100 ft x 100 ft Final Approach and Takeoff (FATO) zones and surfaces capable of handling simultaneous charging for 10+ aircraft.
Regulatory Innovation
Utilities are developing eVTOL-specific rate structures to accommodate unique load profiles. Duke Energy’s NC-AM tariff, approved in January 2025, offers vertiports demand charge exemptions for first-year operations, time-of-use rates with 2.8¢/kWh night-time charging incentives, and shared savings agreements for load-shifting performance. Early adopters using such tariffs report 18–22% lower energy costs than standard commercial rates.
Economic Imperatives and Market Opportunities
According to NREL projections, while often framed as constraints, vertiport power challenges represent a $12–18 billion grid modernization opportunity through 2030. Three areas stand to make substantial gains:
1. Energy Storage Providers
Vertiports’ need for 2–4-hour battery buffers could drive 9.8 GWh of lithium-ion deployments by 2030, a $4.3 billion market. Tesla’s Megapack orders from vertiport developers grew 340% year over year in Q4 2024.
2. Renewable Energy Developers
Offsite power purchase agreements (PPAs) for vertiports are emerging as a financing mechanism for wind/solar projects. Blade Air Mobility’s 72 MW Oklahoma wind PPA covers its Dallas vertiport and sells excess credits into ERCOT’s market.
3. Grid Software Firms
AutoGrid’s VertiMax platform—which optimizes vertiport demand response—has attracted $47 million in venture funding since 2023, reflecting soaring demand for specialized energy management tools.
Global prescriptive
Globally, over 1,500 vertiports are planned by 2025, with significant activity concentrated in Europe and Asia-Pacific. However, regulatory delays could reduce this number to around 980 by 2029. Modular designs are gaining traction due to their adaptability and cost-effectiveness. Harmonizing regulations across regions remains challenging, but initiatives like Europe’s EUREKA project and Singapore’s CAAS-Supernal partnership aim to bridge these gaps.
The global AAM market presents substantial economic opportunities, from China’s low-altitude economy initiatives to Europe’s advanced manufacturing hubs. By fostering international collaboration and addressing shared challenges such as infrastructure readiness and public acceptance, these regions are shaping the future of AAM on a global scale.
In the Asia-Pacific region, AAM is positioned as a key economic driver. China’s Civil Aviation Administration has included AAM in its 14th Five-Year Plan, targeting the development of a “low-altitude economy” with plans to establish over 100 vertiports in Guangdong Province by 2027. This aligns with China’s broader goal of expanding its low-altitude economy to $275 billion by 2030.
Japan is accelerating deployment through subsidies covering up to 50% of vertiport construction costs, with the Ministry of Transport supporting modular designs for airport-based vertiports. Singapore has partnered with Supernal (Hyundai Motor Group) and the Civil Aviation Authority of Singapore (CAAS) to develop regulatory frameworks and public engagement strategies for AAM adoption.
The Middle East is also emerging as a leader in AAM infrastructure development. Abu Dhabi is advancing ambitious projects to establish strategically located vertiports in Al Bateen, Yas Island, and Khalifa Port through collaborations between LODD and Skyports. These facilities will support both passenger eVTOL services and autonomous aerial logistics. The Abu Dhabi Government is actively conducting feasibility studies and regulatory evaluations to ensure seamless integration into its transportation network.
Conclusion:
Ultimately, the success of AAM hinges on treating energy infrastructure as a critical enabler rather than an afterthought. Early engagement with utilities, investments in innovative power solutions, and regulatory modernization will be key to avoiding stranded assets and ensuring operational viability. Beyond aviation, the technologies developed for vertiports can transform energy resilience across industries such as healthcare and data centers. As AAM matures, its legacy may extend beyond urban air transport to redefine how we manage energy in a rapidly electrifying world.